
It has a miraculous property of the chromophore that is responsible for its fluorescence. This chromophore is formed spontaneously from a tri-peptide motif in the primary structure of GFP, so that its fluorescence is automatically turned on in every organism where it is expressed. The maturation of the tri-peptide based chromophore in GFP requires only oxygen and does not depend on the presence of enzymes or other auxiliary factors. GFP and its related variants thereby provide universal genetic tags that can used to visualize a virtually unlimited number of spatio-temporal processes in virtually all living systems. This GFP revolution in the biological sciences has been greatly accelerated by a rapid parallel development of quantitative Light Microscopy, Electronics, Computational power and molecular modelling of intra and inter-cellular processes with systems-biology approaches.
DISCOVERY OF GFP
Davenport and Nicole (1955) first described that the jellyfish Aequorea victoria is bioluminescent, i.e. it produces light with the help of chemical reactions that provide the energy for photon emission and emits green light.
In 1960, Osamu Shimomura joined the laboratory

The active component of the Aequorea bioluminescence was identified as a protein, named aequorin, emitting blue light in a Calcium ion dependent manner (Shimomura et al., 1962). That the light emission of purified aequorin peaked in the blue part of the visible spectrum came as a surprise, since the bioluminescence of Aequorea victoria is distinctly green.

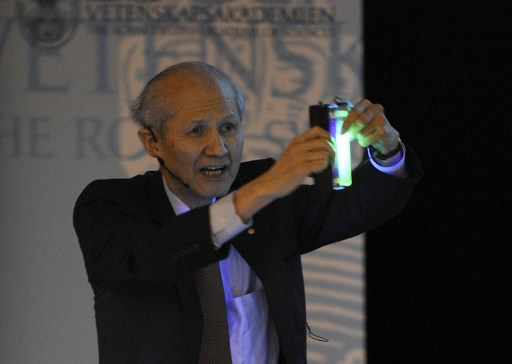
PROPERTIES OF GFP
- The native green fluorescent protein (GFP), first so named by Morin and Hastings, from the jellyfish Aequorea victoria, contains 238 amino acids. Residues 65-67 (Ser-Tyr-Gly) in the GFP sequence spontaneously form the fluorescent chromophore p-hydroxybenzylideneimidazolinone.
- The excitation spectrum of GFP fluorescence has a dominant maximum at about 400 nm and a significantly smaller maximum at about 470 nm, while the emission spectrum has a sharp maximum at about 505 nm and a shoulder around 540 nm.
- The crystal structure of GFP is an eleven-stranded β-barrel, threaded by an α-helix, running up along the axis of the cylinder. The chromophore is in the α-helix, very close to the centre of the can-like cylinder. A very large part of the primary structure of the protein is used to construct the β-barrel and the threading α-helix. The N-terminal residue and the C-terminal residues 230-238, approximately corresponding to the maximal numbers of residues that can be removed from the N- (2 residues) and C-terminal (6 residues) respectively of GFP at retained fluorescence, are disordered and therefore unresolved in this structural image.
- The tripeptide motif Ser65-Tyr66-Gly67- in the primary structure of unfolded or denatured GFP does not display any striking feature. However, as the GFP protein folds into its native conformation, these three amino acids are forced into a sharp turn, greatly favouring a nucleophilic attack of the amide of Gly67 on the carbonyl of Ser65, leading to imidazolinone formation by cyclization and dehydration. At this point, GFP does not fluoresce but, conditional on the presence of molecular oxygen, the α–β bond of residue 66 is subsequently dehydrogenated into conjugation with the imidazolinone, which results in maturation of the GFP chromophore to its fluorescent form.
- GFP is generally non-toxic and can be expressed to high levels in different organisms with minor effects on their physiology (Chalfie et al., 1994). Furthermore, when the gene for GFP is fused to the gene of a protein to be studied in an organism of interest, the expressed protein of interest retains its normal activity and, likewise, GFP retains its fluorescence, so that the location, movement and other activities of the studied protein can be followed by microscopic monitoring of the GFP fluorescence.
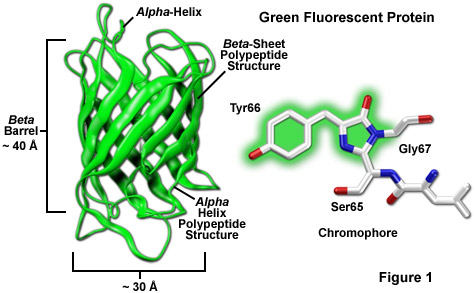
SCIENTIFIC APPLICATIONS OF GFP
- The most common use of GFP has been to monitor the location, movement and chemical reactions involving proteins expressed as fusion partners with GFP. The localization of GFP fusion proteins in different parts of cells, for example during the cell cycle or during exponential growth, has been extensively studied. One example is provided by the pole to pole oscillations of the MinDE system that determine the midpoint of bacterial cells for septum formation and cell division.
- Several spectral variants of GFP tagged to different protein populations in the cell have provided data on how the dynamics of these populations respond to chemical inhibitors, mutations and gene knock outs.
- Another application is provided by monitoring the temporal expression of genes, for example in the formation of large molecular machines like the flagella and their motors, that allow for the chemotactic swimming responses of bacteria.
- By expressing full-length GFP-tagged proteins from their endogenous chromosomal locations at natural levels, it has become feasible to monitor the intracellular location and concentration spectrum of the whole proteasome of different organisms.
- GFP has been used to study membrane-bound organelles, and one key finding is that many of these continuously exchange protein components with each other. For instance, the Golgi apparatus, which receives secretory cargo from the Endoplasmic Reticulum (ER), constitutively recycles its components back to the ER and disassembles duing mitosis.
- In the area of nuclear architectures, GFP-based studies have revealed that interphase nuclear structures are both dynamic and self-organizing.
- GFP fusion proteins have been successfully used to construct sensors of intracellular parameters, like pH or various metabolite concentrations.
- Finally, GFP fusions have been extensively used for imaging cells and tissues within multicellular organisms and have become a very important experimental tool in neurobiology.
No comments:
Post a Comment